Satellite Communications and Space Weather
Introduction
Satellite communication is normally thought of as a robust means of communication, not sensitive to environmental impacts. This perception is not totally accurate. Satellite communication can be and is affected by the environment in which it operates.
Space environmental effects on satellite communication can be separated into (1) effects on the space element (ie the satellite), (2) effects on the ground element (ie the Earth station), and (3) effects on the signals propagating through the Earth's lower and upper atmosphere.
Effects on the satellite depend on the orbit of the satellite. Geosynchronous satellites in the highest orbits are susceptible to bursts of high energy particles that are infrequently emitted from the Sun. These particles may cause (1) memory upsets, (2) dielectric charging and (3) radiation damage to components. This can result in operational glitches, degradation of service or in extreme cases, loss of the satellite.
Satellites in low earth orbits (LEOs) are less susceptible to particle damage, except over the polar regions, but may suffer increased orbital decay (and consequent reduced lifetime) when increased solar activity occurs.
Ground station downlink sensitivity is affected by noise sources in the beam of the receiving antenna. This can include sky noise and solar noise. The effect is dependent on frequency of operation.
The propagating signal may be affected by its passage through the ionosphere (upper atmosphere) or the troposphere (lower atmosphere). These effects depend significantly on frequency, but include signal absorption, scintillation, Faraday rotation and bandwidth decoherence. Geographic location and signal propagation path can determine the extent to which the signal is affected.
The following sections discuss each of the above effects in more detail.
Satellite effects
Space is not the benign environment that was once thought. It is traversed by small pieces of matter (meteoroids) and also by a large and variable radiation flux. The radiation field in Earth orbit comes from three sources, galactic cosmic radiation, trapped radiation belts (the Van Allen belts) and solar radiation. The first two sources are particulate radiation, mostly protons and electrons. Solar radiation is both electromagnetic and particulate (atomic and sub-atomic).
Galactic cosmic radiation consists mostly of very high energy protons that form a constant low level background radiation source. These particles are not particularly significant for communications satellites, but present a possible hazard for long duration manned spaceflights to other planets. Occasionally they may be responsible for memory upsets in communication satellites.
Trapped radiation belts are low energy particulate radiation that must be considered for satellites that spend significant time in medium altitude orbits. The Van Allen belts are in fact responsible for the bimodal distribution of satellites. Orbits below about 1500 km are mostly below the radiation belts, whereas geosynchronous orbits lie above them. Satellites in semi-synchronous orbits (eg GPS satellites) must employ radiation hardened components (particularly in the computer memory area) to survive for many years. So far, Molniya type satellites, with very elliptical orbits, are the only comsats to spend much time in the Van Allen belts, and even these transit the danger region fairly quickly on their way from perigee (where they are non-functional) up to their apogee where they spend most of their active life.
Solar radiation is extremely variable. The background solar ionising radiation consists of low level X-rays and a small particle component we term the solar wind. However, during explosive events that occur on the Sun (flares and coronal mass ejections), the flux of X-rays may increase several orders of magnitude, and the energy of this radiation increases (becomes "harder"). Electrons and protons may be ejected in large numbers, and in rare events some particles may be accelerated to very high energies (even in excess of 1 GeV). It is these solar energetic particles (SEP's) that can cause damage to spacecraft.
SEP's may cause direct radiation damage to spacecraft components such as large solar cell arrays. Some spacecraft have had the efficiency of their solar cells reduced by over 30% in a single large solar particle event. This effectively reduces the lifetime of the spacecraft by several years (and denies the owner several million dollars revenue). Even large numbers of lower energy electrons have caused damage to satellites, in one case resulting in a total spacecraft loss (a Canadian Anik geosat).
Other effects of particles on satellites are related to vehicle charging. In cases where a spacecraft has surfaces which have a small radius of curvature, and in particular several surfaces that may be insulated from each other, differential surface charging can occur. This may induce deep dielectric charging in the space-craft's circuit boards. If the charge builds to a high value, a sudden discharge may occur with resultant damage to electronic components. Single particles may also deposit sufficient charge inside the space-craft near memory cell components and result in a "bit-flip", changing the state of the memory cell from a zero to a one (or vice versa), causing an error in a system program or data. These are temporary effects referred to as single event upsets or SEU's.
A potentially dangerous condition can arise for geosynchronous satellites (geosats) when solar wind and interplanetary magnetic field conditions create sufficient pressure to push the boundary of the Earth's magnetosphere (called the magnetopause) to a lower altitude than the satellites orbit. Normally the magnetosphere, the region where the Earth's magnetic field controls the motion of particles in space, provides a degree of shielding and protection to satellites within its borders. If a satellite finds itself outside the magnetosphere, effectively "in space", then it will be exposed to a massive increase in solar particulate radiation when on the sunward side of the Earth. In addition, older satellites also rely on the Earth's magnetic field to maintain correct orientation. When a magnetopause crossing occurs, these satellites will lose their orientational reference.
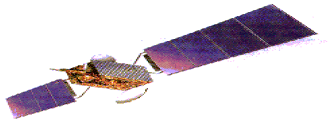
Ground station interference
A satellite ground station is responsible for receiving signals from a satellite and possibly transmitting commands and/or communication material to a satellite. For the reception of satellite signals the ground station employs an antenna to "capture" the satellite signal. All antennas have a beamwidth, an angular range over which they can detect a signal, which is determined by the size or aperture of the antenna and the frequency of operation. The larger the antenna, the smaller will be its beamwidth, and the higher the frequency of operation, the smaller the beamwidth.
Any received signal has to compete with background noise. A parameter known as the signal to noise ratio (SNR) determines whether the antenna receives a useable signal. For normal communications, the power of the signal of interest must be at least 10 dB (a factor of ten) above whatever background noise is present, to be useable.
Noise in a system comes from two sources; internal and external. Every receiver generates some internal noise. This may be minimised by careful design, but cannot be entirely eliminated, and eventually sets a limit on all communication. External noise enters via the receiving antenna, and comes from any sources (other than the desired satellite) that may coincidentally lie within the beamwidth of the antenna. The Sun, with a temperature that may vary from 6000 to 2 million degrees, is a strong source of radio noise, moving across the sky daily and possibly entering the beam of the receive antenna. For geosats this tends to happen around the equinoxes (March and September), when the declination (celestial latitude) of the Sun equals the apparent declination of the geosat. When this occurs, the satellite signal must compete against the solar noise signal. Even at times of low solar activity, this signal is typically about 20 decibels (a factor of 100 in power) above the typical C-band (4 GHz) satellite TV transponder.
All satellite communications are subject to "sun-outages" described above. Systems that have small beamwidths and high SNR's will be most resistant to a sun-outage. Systems with large beamwidths and low signal to noise ratios will be more affected. The Sun's radio noise also increases with frequency, so K band systems will often be at greater risk than C band systems.
Even satellite communication systems which have proven robust on occasions of quiet solar conditions, may be affected during an energetic solar event where the radio noise output at all frequencies can rise by several orders of magnitude.
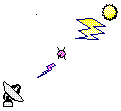
Effects on the propagating signal
Ionospheric Effects
The ionosphere is a region of the upper atmosphere that extends from about 70 to 500 km in altitude. It is a region where some of the atoms have had their outer electrons removed by extreme ultra-violet (EUV) and X-ray radiation coming from the Sun. The atmosphere is thus said to be partially ionised - hence the name, ionosphere. An ionised gas is also referred to as a plasma. A plasma is conductive, and because of this it will interact with electromagnetic signals (eg radio waves) that pass through it. In fact, below a certain frequency (the plasma frequency, which is proportional to the square root of the plasma electron density), a plasma will reflect a radio signal incident upon it. It is this property that allows the propagation of high frequency (HF) or shortwave signals around the globe. Above the plasma frequency, a signal will not be reflected, but will still be refracted or bent as is traverses the plasma. The higher the frequency of the radio signal, the less the bending.
The ionosphere is not a uniform layer of plasma. Its density changes with time of day, altitude, latitude, season and solar activity. It may contain irregularities such as patches, clumps, and troughs of ionisation. It is also a dispersive medium, that is, one through which signals of different frequencies travel at slightly different velocities. At the lowest altitudes it also tends to absorb radio waves rather than reflect them. All these characteristics cause a variety of effects on propagating electromagnetic signals.
Direct absorption at low altitudes and reflection at higher altitudes only occurs for signals below about 30 MHz, so these are not usually a problem for satellite communications. However, refraction (bending) and dispersion are important issues for satellite links. In a uniform ionosphere, refraction is an important consideration for radars tracking space objects as it causes them to see the object in a position displaced from the true one. This is the same as looking at an object immersed in water which appears displaced from where it really is, due to the refraction (bending) of light. Dispersion causes signal delay and differential delay in wideband communication systems that can be a problem. Another phenomenon, the Faraday effect, occurs when a signal propagates through a plasma in the presence of a magnetic field. The result is a rotation of the plane of polarisation of a plane polarised signal.
When the ionosphere contains irregularities, we are faced with the phenomenon of scintillation. This effect is considered the following section.
Scintillation
Ionospheric scintillation is a rapid fluctuation in the signal strength of a trans-ionospheric signal (eg from satellite to ground station). Effectively scintillation introduces an additional low frequency noise component on the signal.
Scintillation, which is similar to the visible twinkling of stars in the night sky, is caused by small-scale irregularities in the ionosphere. That is, instead of a uniform layer of ionisation, certain regions of the ionosphere are subject to patches of lower or higher density ionisation.
These irregularities preferentially form in two different regions over the Earth - the polar, or more correctly the auroral regions (both north and south), and the equatorial regions. In the polar regions, ionospheric irregularities are caused by particles precipitating down into the ionosphere from the magnetosphere (the same particles that produce the visible aurora). Flows of these particles cause bubbles and troughs which are not stable and at whose edges scintillations are the strongest. Auroral scintillations may occur at any time of day, but tend to be stronger at night, and when geomagnetic activity is high (ie during geomagnetic storms). In the equatorial regions, after sunset, bubbles of ionisation form at the bottom of the ionosphere and rise upward during the night forming vertical plumes (which can also be moving horizontally). Signals that propagate near the edges of these plumes are subject to the most intense scintillations. Equatorial scintillations are thus basically a night-time phenomenon, with most of the plumes disappearing by midnight, although some do persist into the early morning hours. Equatorial scintillations increase in strength as the Sun's extreme ultra-violet (EUV) and X-ray output increases (which produces a thicker and more strongly ionised ionosphere). Thus their intensity follows the approximately 11 year solar cycle. They also display a 27 day periodicity due to the solar rotation, since the EUV producing plage is distributed unevenly across the solar surface.
Both the phase and intensity of a trans-ionospheric signal are affected by scintillations. Intensity fluctuations, which may occasionally be large enough to cause deep signal fades, are not caused by signal absorption within the ionospheric irregularities, but rather by a phase change of various parts of the signal wavefront. Constructive and destructive interference of various signal paths as observed on the ground, produce the observed changes in signal strength.
It is equatorial scintillations that are of most significance for geosynchronous satellites. These are found to be greatest within 20 degrees north and south of the geomagnetic equator, which is close to the geographic equator. A signal that transits the ionosphere at a geomagnetic latitude of around 15 to 20 degrees will be most affected. The geomagnetic equator changes as a function of longitude. Over parts of the world from the middle east to Australia, the geomagnetic equator is well north of the geographic equator. Over the Americas, the geomagnetic equator is well south of the geographic equator, as shown in the world maps below.
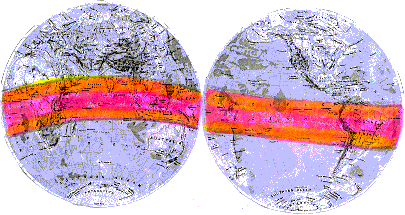
The shaded areas show the approximate regions that are generally affected by medium to strong scintillations. The most intensely affected regions lie toward the north and south edges of the shaded areas.
To determine whether a particular signal path will be affected by ionospheric scintillation, it is necessary to determine the geomagnetic latitude of the ionospheric penetration point (IPP) of the trans-ionospheric signal. The table below shows the IPP for signals received at Darwin (Australia) from a range of geosynchronous satellites. Note that many of these are close to 20 degrees south (geomagnetic), and will thus be subject to ionospheric scintillation, which will increase to the east and also toward solar maximum as overall electron density increases. Scintillation effects, when they occur, will become apparent just after local sunset, and will diminish after local midnight. By 3 am local time, all signal scintillations should have disappeared.
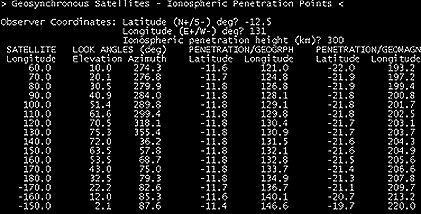
Ionospheric scintillations are worse at lower frequencies than high. VHF frequencies, such as the 240 MHz frequencies used in military communications, suffer the most, while L-band is moderately affected, and only the strongest scintillations affect C-band and above.
Faraday Rotation
When a plane polarised radio signal travels through a plasma, such as the ionosphere, in which a magnetic field (such as the Earth's magnetic field) is also present, the plane of polarisation of the signal is rotated. The amount of rotation is proportional to the magnitude of the magnetic field and the total ionisation through which the signal passes. It is also inversely proportional to the square of the frequency of the signal.
Low frequencies thus suffer significantly greater rotation than high frequencies. As the ionosphere becomes more ionised, due to increasing solar EUV and X-ray flux, signal rotation also increases. Signals traversing the ionosphere at low elevation angles will be affected more than signals propagating near the zenith, because the total distance traversed through the ionosphere will be greater.
At VHF frequencies and high solar activity levels, the plane of polarisation may be rotated through many times 360 degrees. At C-band frequencies (4 GHz) the signal rotation will rarely exceed a few degrees.
The problem caused by a rotation of the plane of polarisation of a signal is two-fold. If a ground station is expecting to receive a horizontally polarised wave, then a 90 degree rotation could result in a total loss of signal. However, much smaller rotations can cause problems in satellite communication systems that use both vertically and horizontally polarised signals of the same frequency (ie, spectral re-use through orthogonal polarisations). In this case a small rotation will barely affect the received signal strength of the desired polarisation, but it will allow some of the orthogonally polarised signal (the undesired channel) to cross-couple into the antenna. This results in interference to the desired signal. A rotation as small as 5 degrees may couple in the undesired channel to a level only 20 dB below the desired channel.
The graph below shows a typical variation of ionospheric total electron content throughout a day of high background solar activity, the resulting Faraday rotation that is produced in a C-band satellite TV downlink, and the orthogonal power ratio coupling that results. The scale on the right indicates the ratio of the cross-coupling interference to the main signal, in this case reaching around -20 dB (or a ratio of 1 to 100).
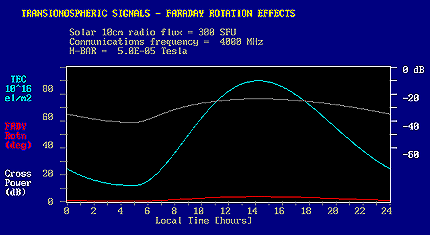
Tropospheric Absorption and other Effects
It is not only the upper atmosphere that can cause problems with satellite communication links. Tropospheric weather conditions, "conventional" weather occuring in the lower 10 km of the atmosphere, can also cause losses in signals propagating between satellite and ground stations.
Water vapour is particularly damaging to signals above about 2 GHz, causing absorption of signals which becomes greater as the frequency increases. K-band signals (10-20 GHz) are particularly susceptible, and precipitation in the vicinity of satellite ground stations can cause total loss of signal. Again, signals with low elevations are more affected than those propagating near the zenith, because the wave has to follow a longer path through the atmosphere.
At frequencies above 20 GHz we start to encounter resonant absorption at specific frequencies. Oxygen, in particular, will absorb electromagnetic energy only at certain well-defined frequencies. These frequencies correspond exactly to the energies required to lift the Oxygen atoms into higher energy states. Satellite communication links are designed to avoid these well known frequency bands.
Significance summary
- Damage to a communications satellite may occur following a solar energetic particle event such as a Coronal Mass Ejection (CME). Damage could be anything from a momentary glitch through to significant subsystem damage, reduced satellite lifetime or complete loss of the satellite. Generally, the larger the solar particle event the greater the damage.
- When the Sun lies in the beam of the satellite ground station, the satellite signal will experience a reduced signal to noise ratio. For geosynchronous satellites the problem occurs regularly around the time of the equinoxes, and may cause complete loss of signal for a period ranging from a few minutes to an hour or so. The "sun-outage" effect can be significantly increased during periods of high solar radio burst activity.
- Satellite communications using lower frequencies (VHF through L-band) can experience significant short term signal losses (dropouts) due to ionospheric scintillations. The problem is minimal when the signal path lies in the mid-latitudes, but can become severe when the signal passes through either the equatorial ionosphere or the polar ionosphere. Equatorial scintillations are particularly bad in certain parts of the world (eg Arabian Gulf), and will be important for northern Australian stations communicating via a geosynchronous satellite. Such equatorial scintillations affect systems mostly after sunset until a few hours after midnight. Increased solar and geomagnetic activity increase the severity of the effects. Wideband systems are more affected than narrowband systems.
- Satellite systems using linear polarisation multiplexing (horizontal/vertical) can experience signal depolarisation due to Faraday rotation of the signal as it passes through the ionosphere. The effect is more severe at the lower (VHF) frequencies, but at times of high solar activity even C-band systems may experience an effect. The effect is to reduce the signal strength of the desired signal and to cross couple power from the undesired (orthogonally polarised) channel into the desired channel.
- Higher frequency signals (X, K and higher bands) are significantly affected by lower atmosphere (troposphere, < 10km altitude) constituents such as clouds, fog, dirt, sand and severe air pollution. Precipitation in the propagation path can cause severe signal attenuation to the point of complete signal loss. Higher frequencies are affected more than the lower bands. At certain discrete frequencies, resonanant absorption by atmospheric atoms occurs. These frequencies need to be avoided.
Mitigating space weather effects
There are a number of strategies that may be taken to minimise the effects of space weather on satellite communication systems.
As far as the satellites themselves are concerned, the first step should occur in the design phase prior to launch. Satellite design can minimise the potential for spacecraft charging, and the consequent discharging that may cause damage to components. This is done by choice of materials, surface contours and adequate "ground" bonding.
Once in orbit, an astute satellite operator may be able to orient a satellite to minimise damage from high energy particles (mostly protons) resulting from a SEP event, given adequate warning by the appropriate space weather agency. A satellite controller might also choose not to upload commands during such events. To maintain satellite attitude, particularly in LEOs, the controller might wish to perform a "momentum dump" to make sure that the satellite reaction wheels are not at maximum rpm when the disturbance arrives.
Sun-outage effects can only be mitigated by using more than one satellite, at different orbital locations. Traffic might be temporarily redirected to the satellite which is not in front of the Sun at the specific time. Such "spatial diversity" might also be applied to tropospheric absorption to avoid local regions of high precipitation.
Ionospheric scintillations occur at particular times of the day and it might be possible to schedule traffic so that peak demand does not occur during the evening hours, or to use an alternative link during this time.
Signal depolarisation can be overcome by rotating the antenna feed to compensate for the changing rotation produced by the Faraday effect. An automatic adaptive system might be employed either by the ground station or by the satellite controller who monitor the effect with a beacon transmission from the satellite in question.
Even if it is not possible or feasible to take definitive action to eliminate a problem associated with space weather, it is always important for satellite operators to know whether a problem has been caused by a space weather effect or from a hardware or software failure.
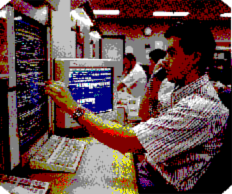
Material prepared by John Kennewell and Andrew McDonald